Beyond Vogtle: Future of Nuclear Energy with Small Modular Reactors
Conflicting trends for nuclear power in the United States emerged in 2023. Among the positives was the completion in July, after years of delay, of Vogtle 3, the first built-from-scratch nuclear unit constructed in the United States in decades. In December, at the COP 28 climate conference in Dubai, the United States was one of 22 signatories to a declaration that recognized the “key role” of nuclear energy in achieving net zero climate emissions. The declaration established an “aspirational” goal of tripling world nuclear capacity by 2050. Nuclear power now has a prominent seat at the climate roundtable.
But other developments in the United States and Europe were less encouraging. Commercial operation of Vogtle 4, the twin of unit 3, was delayed yet again to first quarter 2024. When finally completed, Vogtle 3 and 4 will be seven years late and about 100 percent over budget with a total cost of about $35 billion. The story in Europe is not better, where four new reactors have been under construction. In Finland, Olkiluoto 3 entered commercial service in April 2023, 17.5 years late, and in France, the Flamanville 3 unit is now 12 years behind schedule and still under construction. In the United Kingdom, commercial operation of the two-unit Hinkley Point C plant – a mammoth 3200 MW project – has been delayed from 2025 to at least 2029, and the cost has risen from $27.6 billion to north of $44 billion.
This history of lengthy delays and spectacular cost overruns make it increasingly unlikely that traditional large (1000 MW-class) nuclear power plants will be built any time soon in the United States. But there is an alternative, the comparatively diminutive Small Modular Reactor (SMR). SMR technology has also faced headwinds but is arguably still the best chance for new nuclear construction in the United States in the near term. This blog post provides an overview of SMR technology, economics, and developments.
What is an SMR?
An SMR is notionally a reactor with a size of 10 to 300MW. A model SMR project entails building multiple units at one site, perhaps phased over several years. Instead of the bespoke field construction required by large nuclear (or, for that matter, coal) plants, in a modular approach the major components are built and assembled in factories and shipped to the construction site. In principle modular construction reduces costs, facilitates quality control, and speeds the schedule. The underlying nuclear technology can be a light water design, typical of most operating reactors, or advanced “Generation IV” technology that makes use of more exotic cooling mediums that operate at high temperatures, such as helium gas or molten sodium.
The small size is the single most important feature of the SMR. Reduced size facilitates modular construction and assembly, and makes a multi-unit project into a series of modest construction efforts instead one massively complex undertaking, reducing schedule risks. SMRs can make use of integral design, an approach that integrates more of the system components directly into the reactor vessel and arguably improves safety. Because of the reduced size, the cost of each reactor is lower and carries less financial risk than the bet-the-company cost of traditional large reactors.
Because of these attractive features there has been a lot of interest in SMRs. There are currently around 70 proposed designs. There are plans – on paper – for SMR projects in locations as diverse as Pennsylvania, Canada, and Romania. But to date not much ground has been broken. There are two active SMR projects of which one is operational, the 75 MW Akademik Lomonosov Russian floating reactor. In China, construction is advanced on the prototype 125 MW Linglong One plant, which is scheduled to enter service in 2026. In the United States, the flagship SMR effort, the Carbon Free Power Project in Idaho, was canceled in November 2023. The schedule had slipped, costs had ballooned, and it failed to attract enough subscribers to be viable.
The SMR concept dates back decades, and with the travails of large reactor projects in recent years interest has only grown. Why then has there been so little progress? As discussed below, size is once again the driving force.
Nuclear Economies and Diseconomies of Scale
The principal factor that determines the cost of electricity from a nuclear power station is the capital cost of constructing the plant. While fuel and operating costs are not immaterial, capital cost is the most important driver. This has led developers to seek economies of scale by building plants with a large generating capacity, so that recovery of the fixed capital costs can be spread over the output of many kilowatt-hours (kWh) of electricity. An example of this approach is the European Pressurized Reactor, a 1,600 MW goliath which is the type being built at Olkiluoto, Flamanville, and Hinkley Point C.
As discussed above, the construction of large reactors in the United States and Europe has backfired, with cost and schedule overruns. This has led to the interest in building smaller, simpler reactors. But the problem with a 300 MW, much less a 10 MW, reactor is diseconomies of scale. The capital costs of building even a small nuclear station are still significant, and these plants produce far fewer kWh over which the fixed costs can be recovered.
Developers have struggled to find the right balance between the theorized benefits of smaller size and the economic penalties. This is illustrated by the repeated efforts by Westinghouse to find the nuclear sweet spot. In the 1990s it promoted a new 600 MW design, the AP600, but it had no takers. It then upgraded the technology to produce the AP1000 design, with a capacity of 1100 MW. This is the design that was constructed, with the problems noted earlier, at Vogtle. Most recently the company is trying to interest buyers in the AP300, yet another variation of the same technology that it is marketing as an SMR.
Another consequence of the diseconomies of scale problem is what might be termed “capacity creep” in the SMR field. Some SMR designs test the limits of what might be termed “small”; Rolls Royce is marketing a design with a capacity of 470 MW that it terms an SMR. In other cases designs have evolved toward greater capacity. South Korea has moved from a 100 MW to 170 MW design; NuScale has increased the output of its design from 50 MW to 77 MW; the TerraPower design promoted by Bill Gates has a nominal output of 345 MW but uses integrated molten salt storage to achieve peak output of 500 MW. The reason for capacity creep is put plainly by Rolls Royce: “its Chief Technical Officer traced the increase to a desire ‘to minimize the cost of energy coming out… the cost being the historical challenge of nuclear power.’”
Clearly the size of SMRs can only be increased so far before the benefits of smaller size are lost and the SMR concept becomes meaningless. The other path that proponents of SMRs have suggested for reducing costs might be termed economies of mass installation. The idea is that once dozens of a single design have been built and installed, a combination of learning by doing and savings through the mass production of standardized reactor modules from factories will drive down costs to competitive levels. The economies of mass installations would be enhanced through international harmonization of the rules and regulations for building and operating an SMR (see Figure 1).
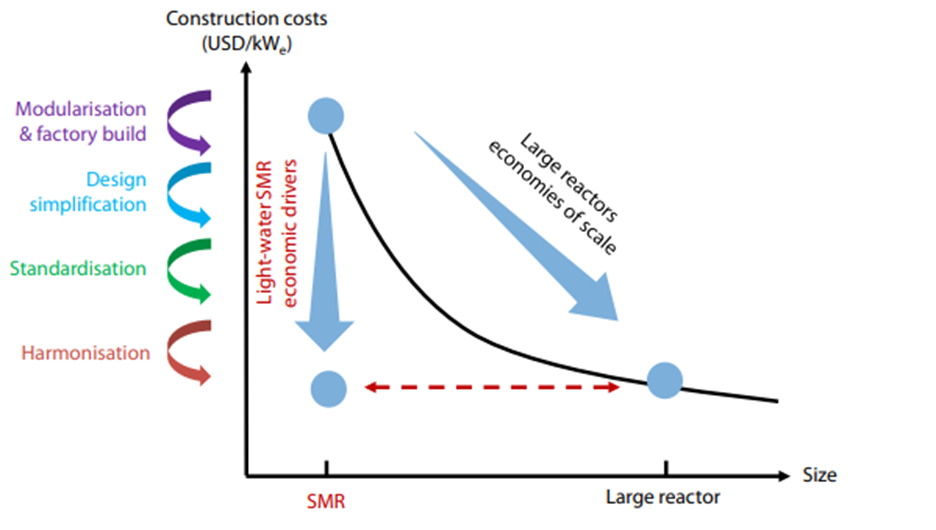
Figure 1. Driving down SMR costs through mass production and standardization. (Source: Nuclear Energy Agency)
Looking Far Afield
There is an obvious chicken and egg problem with this approach – if dozens of reactors have to be built before SMR costs become competitive, where are the first movers who will pay to install the costly initial batch of reactors? This is where government subsidies come into play, a subject we will return to in the conclusion of this blog. Another solution is to search for niches in which SMRs can provide a carbon free solution and the high cost will be less of a concern. SMR proponents have suggested such applications as:
- Small reactors for cogeneration, district heating, and industrial applications that require high temperature heat. This is where advanced reactor concepts that operate at high temperatures, such as the TerraPower liquid sodium design, come into play.
- Isolated, off-grid locations, such as remote mining sites, where even fossil fuel generation is expensive to install and supply.
- Nations with low power demand and an underdeveloped transmission grid that cannot handle large, 1000 MW-class reactors.
- Reactors to supply energy for water systems, especially desalination.
- Reactors for hydrogen production, as part of a carbon-free hydrogen economy.
- Nuclear powered cargo ships.
- Countries with a high-priced carbon market and uncompromising goals to reduce carbon emissions
The Akademik Lomonosov, which is intended to provide power to isolated communities, is an example of a niche approach. Whether there are enough of these niches to jumpstart an SMR industry is unclear. Also unclear is how to address the safety concerns associated with installing potentially dozens or even (in the hopes of proponents) hundreds of reactors in isolated locations and places without the technical infrastructure normally used to support nuclear power.
Dealing with Uncertainty
With these economic headwinds, and given the early stage of technology development, entities promoting SMRs have not been shy in stating that government subsidies will be needed to launch the industry, even including the feed-in tariffs usually associated with renewables. According to one estimate, for a single SMR design to become cost competitive will require 50 to 100 deployments of that design. To achieve this will require massive government aid, about $150 billion compared to a total investment of $800 to $900 billion to build 150-160 GW of SMR capacity globally by 2050.
This creates a highly uncertain situation – what kind and level of subsidies will be the most effective, accounting for cost and schedule uncertainty, in supporting the creation of an SMR industry? This has implications for national industrial policy as well as nuclear and climate policy. If it will indeed take 50 or more installations of a single design to make that design cost competitive, then even the global market for SMRs will probably support only a handful of designs. In 2019 the Biden Administration issued an executive order stating that “A healthy and robust nuclear energy industry is critical to the national security, energy security, and economic prosperity of the United States… The United States should maintain technology supremacy for nuclear research and development, manufacturing proficiency, and security and safety. (emphasis added).” The Bipartisan Infrastructure Law of 2021 authorizes $3.2 billion for DOE’s Advanced Reactor Demonstration Program, and the Inflation Reduction Act of 2022 includes production tax credits and investment tax credits that can be used to support new nuclear construction. (Both acts also include subsidies to keep existing nuclear plants operating.) But more may be needed to support a robust American nuclear industry.
In determining what mix of subsidies and other policies can best achieve these goals, modeling can play an essential role. OnLocation offers NEMS, for decades the standard model for studies of the U.S. energy economy. Analysts can use NEMS to explore alternative policy and financial approaches for supporting the American SMR and general nuclear industries. Different scenarios for SMR cost and performance, and for macroeconomic and federal policy variables, can be examined to help recommend preferred approaches and better understand the possible range of future outcomes.